Author: Ronen Shekel
Even the most stable laser isn’t perfectly quiet. The fact that light comes in discrete photons introduces statistical fluctuations known as shot noise, leading to the standard quantum limit for optical measurement precision.
However, quantum optics provides a workaround. A squeezed state redistributes uncertainty, shrinking noise in one property (phase or amplitude) below the shot‑noise floor while increasing it in the other. On a phase-amplitude diagram the round noise circle turns into an elongated ellipse. Such engineered low-noise light boosts the sensitivity of LIGO’s gravitational‑wave detectors [1], squeezed‑light magnetometers [2], and many other quantum sensing and communication applications.
A common method to generate squeezed light is through a Degenerate Optical Parametric Amplifier (DOPA). In a DOPA, a strong pump laser beam interacts with a nonlinear crystal, typically placed inside an optical cavity to enhance the interaction, and drives the generation of photon pairs. The phase‑sensitive nature of the process allows the cavity to reduce fluctuations along one quadrature while amplifying the orthogonal one. The strength of this interaction is characterized by its effective nonlinear coefficient; a higher value usually indicates a more efficient conversion process, which can translate to stronger squeezing for a given crystal length and pump power.
The Crystal Choice: Operating Temperature and Intrinsic Noise
Two prominent nonlinear materials for constructing DOPAs are periodically poled Potassium Titanyl Phosphate (PPKTP) and periodically poled Lithium Niobate (PPLN). PPLN has a higher nonlinear coefficient than PPKTP, suggesting it is inherently better for generating strong squeezing. However, practical operational requirements introduce crucial differences.
PPLN often suffers from a photorefractive effect when exposed to high-intensity laser light near room temperature. This effect involves the light-induced generation and migration of charge carriers within the crystal, creating internal electric fields that distort the crystal’s refractive index and degrade the quality of the transmitted laser beams. To mitigate photorefraction, PPLN-based devices are typically operated at high temperatures, such that the trapped charges have enough energy to become mobile again, averaging out the internal fields and mitigating the effect. In contrast, PPKTP-based DOPAs can operate efficiently near room temperature without significant photorefractive problems.
This difference in operating temperature has significant consequences for the ultimate noise performance. At elevated temperatures, the atomic lattice vibrates, resulting in microscopic acoustic waves propagating through the crystal. These waves randomly modulate the refractive index. This phenomenon, known as Guided Acoustic Wave Brillouin Scattering (GAWBS), introduces a fundamental source of phase noise that is directly dependent on the crystal’s temperature [3].
A direct experimental comparison by Wan et al. highlighted this critical trade-off [4]. Using identical 1550 nm DOPA setups, they found 6.8 dB of squeezing – that is, 6.8 dB of noise reduction below the standard quantum limit – for room-temperature PPKTP versus 4.9 dB using PPLN at 135°C. The superior squeezing performance of PPKTP, despite its lower nonlinear coefficient, was directly attributed to the reduced GAWBS-induced phase noise at its cooler operating temperature.
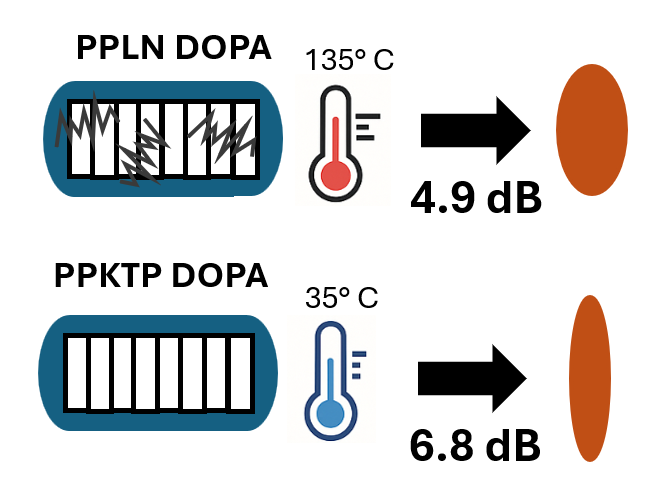
For the strongest phase squeezing, eliminating GAWBS matters more than the absolute value of the nonlinear coefficient. Indeed, record squeezing levels of 12 dB at 1550 nm and 15 dB at 1064 nm both used PPKTP [5, 6], underlining its exceptional low-noise properties.
Raicol: Precision Crystals for Quantum Light
Deep squeezing requires crystals with precisely controlled poling, ultralow absorption, and exceptional uniformity. Raicol’s PPKTP crystals are engineered to these exacting standards, with options for custom periodicities and aperiodic poling designs. For your next squeezed light source, we invite you to explore our products and consult with our team to identify the optimal crystal configuration for your specific applications.
References
[1] Aasi, Junaid, Joan Abadie, B. P. Abbott, Richard Abbott, T. D. Abbott, M. R. Abernathy, Carl Adams et al. “Enhanced sensitivity of the LIGO gravitational wave detector by using squeezed states of light.” Nature Photonics 7, no. 8 (2013): 613-619.
[2] Wolfgramm, Florian, Alessandro Cere, Federica A. Beduini, Ana Predojević, Marco Koschorreck, and Morgan W. Mitchell. “Squeezed-light optical magnetometry.” Physical review letters 105, no. 5 (2010): 053601.
[3] César, Jônatas Eduardo da Silva, A. S. Coelho, Katiuscia Nadyne Cassemiro, Alessandro de Sousa Villar, M. Lassen, P. Nussenzveig, and Marcelo Martinelli. “Extra phase noise from thermal fluctuations in nonlinear optical crystals.” Physical Review A—Atomic, Molecular, and Optical Physics 79, no. 6 (2009): 063816.
[4] Wan, Zhenju, Jinxia Feng, Yuanji Li, and Kuanshou Zhang. “Comparison of phase quadrature squeezed states generated from degenerate optical parametric amplifiers using PPKTP and PPLN.” Optics express 26, no. 5 (2018): 5531-5540.
[5] Mehmet, Moritz, Stefan Ast, Tobias Eberle, Sebastian Steinlechner, Henning Vahlbruch, and Roman Schnabel. “Squeezed light at 1550 nm with a quantum noise reduction of 12.3 dB.” Optics express 19, no. 25 (2011): 25763-25772.
[6] Vahlbruch, Henning, Moritz Mehmet, Karsten Danzmann, and Roman Schnabel. “Detection of 15 dB squeezed states of light and their application for the absolute calibration of photoelectric quantum efficiency.” Physical review letters 117, no. 11 (2016): 110801.
Do you have a question? Our experts will be happy to hear from you and advise you on the best product for you. Contact Us.
Have you already subscribed to our YouTube channel? Don’t miss out—subscribe now for exclusive content and updates from our company.
Author: Ronen Shekel
Building large-scale quantum networks hinges on connecting different quantum systems, but these often operate at distinct optical wavelengths. Nitrogen-vacancy centers in diamond, for instance, are excellent candidates for quantum network nodes, emitting single photons at 637 nm [1].
However, transmitting these photons efficiently over long distances through standard optical fibers is problematic, as 637 nm light suffers significant loss. Conversion to the low-loss telecommunication bands is therefore essential.
Quantum frequency conversion (QFC) provides the solution, coherently shifting the wavelength of quantum light while preserving its crucial quantum properties, like entanglement or squeezing. Despite progress, achieving efficient QFC without introducing significant noise has remained a major challenge [2].
Even small amounts of noise can degrade or destroy the fragile quantum states, severely limiting quantum communication and networking capabilities.
A promising new platform, recently demonstrated by Mann et al. [3] using a crystal supplied by Raicol, utilizes a monolithic bulk ppKTP cavity. A “monolithic” cavity means the reflective mirrors forming the optical resonator are coated directly onto the crystal’s end facets, creating a single, integrated device.
This innovative design dramatically boosts the effective power of a modest, off-the-shelf 1064 nm pump laser circulating inside it. The enhanced pump power then efficiently drives the difference frequency generation (DFG) process needed to convert 637 nm photons to the telecom C-band (around 1587 nm in their work), while keeping the generation of unwanted noise photons remarkably low (Fig. 1).
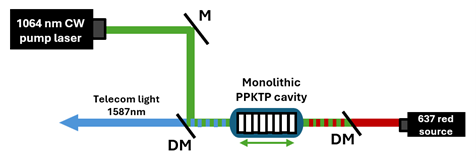
The Power of Monolithic Cavities
Efficient QFC in bulk crystals typically requires high pump powers, often necessitating expensive and complex laser systems. Resonant cavities offer a clever workaround, building up intense light fields inside the crystal from modest input powers. The monolithic design offers specific advantages: it is inherently compact and robust. Furthermore, these cavities can exhibit passive thermal self-tuning [4], meaning they can naturally stay on resonance without needing active electronic feedback loops.
This simplification significantly enhances reliability and practicality for real-world network deployment. Mann et al. [3] also hypothesize that a key advantage comes from using bulk ppKTP: the high quality of periodic poling achievable in bulk KTP [5] may intrinsically suppress parasitic nonlinear processes, particularly unwanted spontaneous parametric down-conversion (SPDC) originating from the strong pump – a major noise source in other QFC approaches.
Record Low Noise and Quantum State Preservation
The Path Forward
The Mann et al. experiment achieved an impressive internal conversion efficiency of up to 72% using only a 3 W input pump laser. Even more significantly, the noise generated was exceptionally low, measured at approximately 110 kHz/nm – a figure quantifying the rate of unwanted noise photons generated per nanometer of bandwidth at the target wavelength. This result represents a 5-fold noise reduction compared to the previous best state-of-the-art single-step converters operating at these wavelengths, dramatically increasing the feasibility of realistic quantum communication links.
Preserving the quantum nature of the light through the conversion process is crucial. The team rigorously verified this by converting single photons from an entangled pair source (SPDC). Measurements of the second-order correlation function confirmed the preservation of non-classical correlations, showing behaviour far exceeding classical limits. They also performed Franson interferometry and a chained Bell inequality test, demonstrating convincingly that time-energy entanglement was preserved with high fidelity through the conversion process.
This work highlights the significant potential of monolithic bulk ppKTP cavities as a robust, efficient, and remarkably low-noise platform for quantum network wavelength conversion. At Raicol Crystals, we provide the high-quality nonlinear crystals essential for this work, including ppKTP, apKTP, and monolithic PPKTP with broadband mirror coatings.
Our expertise in precise periodic and aperiodic poling techniques enables researchers to achieve optimal results in quantum optics applications. We also collaborate with research teams to develop customized monolithic designs that maximize efficiency while maintaining ultra-low noise profiles.
Our ongoing development of narrowband photon sources represents our commitment to advancing quantum technology. Contact Raicol Crystals to explore how our crystals can support your quantum research objectives and help bridge critical wavelength gaps in your experimental systems.
References
[1] Ruf, Maximilian, Noel H. Wan, Hyeongrak Choi, Dirk Englund, and Ronald Hanson. “Quantum networks based on color centers in diamond.” Journal of Applied Physics 130, no. 7 (2021).
[2] Dréau, Anaïs, Anna Tchebotareva, Aboubakr El Mahdaoui, Cristian Bonato, and Ronald Hanson. “Quantum frequency conversion of single photons from a nitrogen-vacancy center in diamond to telecommunication wavelengths.” Physical review applied 9, no. 6 (2018): 064031.
[3] Mann, Felix, Helen M. Chrzanowski, Felipe Gewers, Marlon Placke, and Sven Ramelow. “Low-noise quantum frequency conversion in a monolithic cavity with bulk periodically poled potassium titanyl phosphate.” Physical Review Applied 20, no. 5 (2023): 054010.
[4] Zielińska, Joanna A., and Morgan W. Mitchell. “Self-tuning optical resonator.” Optics letters 42, no. 24 (2017): 5298-5301.
[5] Mann, Felix, Helen M. Chrzanowski, and Sven Ramelow. “Low random duty-cycle errors in periodically poled KTP revealed by sum-frequency generation.” Optics Letters 46, no. 13 (2021): 3049-3052.
Have you already subscribed to our YouTube channel? Don’t miss out—subscribe now for exclusive content and updates from our company.
Author: Ronen Shekel
Spontaneous parametric down-conversion (SPDC) is a cornerstone of quantum optics, enabling the generation of entangled photon pairs with remarkable properties. One such property, dispersion cancellation, reveals how entanglement can mitigate effects that typically distort light as it travels through materials. This phenomenon, first explored in the early 1990s, offers both fundamental insights into quantum mechanics and practical applications in quantum technologies. In this post, we discuss how dispersion affects light, how entanglement counters it, and key experiments that have shaped our understanding.
Classical Dispersion: The Spreading of Light
When a short pulse of classical light travels through a vacuum, it retains its compact shape. However, propagation through a material like glass introduces a phenomenon known as dispersion. In a material, the refractive index varies with wavelength, n≈n0+β(λ-λ0 ), where β is the dispersion coefficient at a reference wavelength λ_0, causing different wavelengths of light to travel at different speeds. Since a short pulse comprises a range of wavelengths – owing to its Fourier composition – each component propagates at its own velocity. As a result, the pulse broadens over time.
In materials exhibiting normal dispersion, longer wavelengths (lower frequencies) tend to move faster, while in those with anomalous dispersion, shorter wavelengths (higher frequencies) take the lead. In either case, the outcome is the same: the initially sharp pulse spreads out, losing its compact temporal profile. This effect poses challenges for applications requiring precise timing or short pulses.
Franson’s Nonlocal Dispersion Cancellation
Consider now a pair of spectrally entangled photons generated via SPDC in a nonlinear crystal. A narrowband pump beam splits into two photons – signal and idler – whose frequencies are tightly correlated due to energy conservation (Fig. 1). When measured jointly, their arrival-time correlations are related to the temporal width of their wave-packets, set by their spectral bandwidth via the Fourier transform. But what happens when these photons travel through dispersive media, where dispersion smears each wave-packet and broadens their correlations?
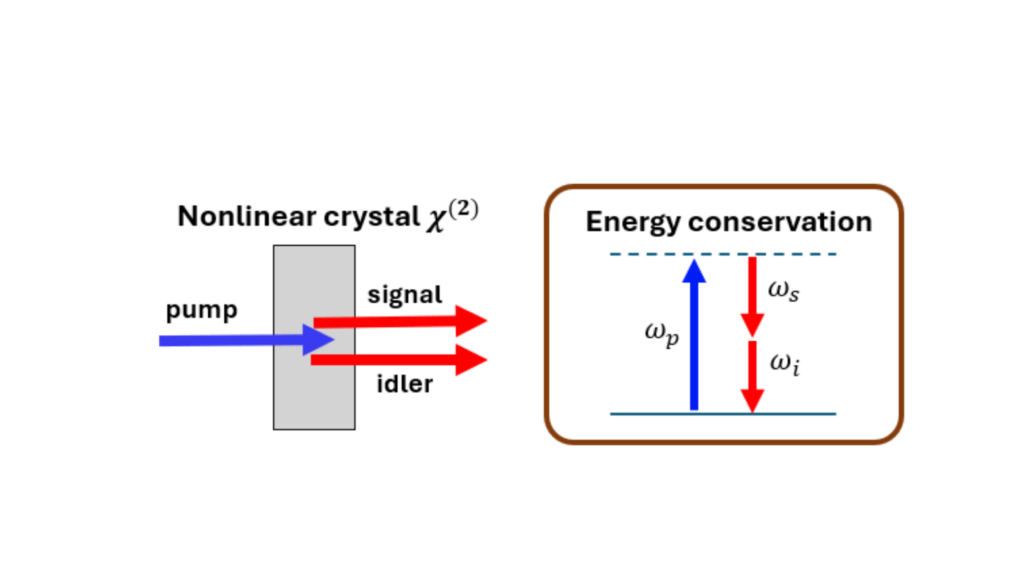
In 1992, J.D. Franson proposed an intriguing experiment (Fig. 2a). He suggested sending each photon through a different dispersive medium – one with normal dispersion, the other with anomalous dispersion – and then measuring their joint arrival times. Classically, one might expect the dispersion to broaden each photon’s wave-packet, resulting in a wider correlation profile. Surprisingly, Franson found that when the dispersions are opposite in sign, the broadening cancels out. The correlation remains as narrow as it was without dispersion!
This effect arises from the entangled nature of the photon pair. The joint two-photon state ensures that the dispersion experienced by one photon is precisely counteracted by that of its twin, a phenomenon described as nonlocal because the photons need not interact directly, and the two detectors might be very far away from each other. While classical analogs to this experiment exist [2], this cancellation highlights a distinctly quantum feature tied to entanglement, as the photons’ individual distortions are reconciled only through their shared state [3].
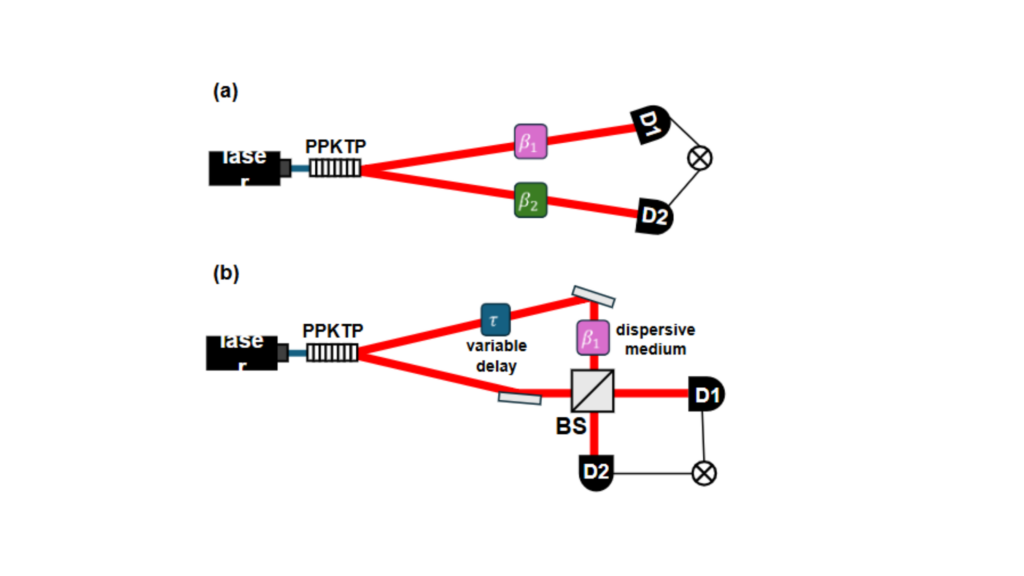
Steinberg’s Local Dispersion Cancellation
Shortly after Franson’s work, Aephraim Steinberg and colleagues proposed a complementary approach [4, 5]. In their experiment, only one of the entangled photons passes through a dispersive medium, while the other travels freely. The photons are then reunited at a beam splitter in a Hong-Ou-Mandel (HOM) interferometer (Fig. 2b), where identical photons exhibit a characteristic dip in coincidence counts due to destructive interference. Specifically, if two indistinguishable photons arrive at the beam splitter simultaneously, they will both exit in the same output port, thereby reducing coincidences and producing the HOM dip.
Once again, one might anticipate that dispersion would stretch the affected photon’s wave-packet, thereby reducing the overlap with its twin and broadening the HOM dip. However, Steinberg showed that the dip remains narrow, largely unaffected by the dispersion. This occurs because the two-photon amplitudes along possible paths through the beam splitter interfere in a way that cancels the dispersion’s impact. Remarkably, this setup also provided experimental evidence that a single photon travels through a material at its group velocity – the speed of the wave-packet’s peak – resolving a point of contention in photon propagation studies at the time.
Developments Since the 1990s
Since these pioneering experiments, dispersion cancellation has been explored in multiple contexts. The Franson effect has been demonstrated using diverse measurement methods [6, 7], and the dispersion cancellation concept has been extended to systems such as Mach-Zehnder interferometers [8] and optical cavities [9]. It has also been generalized to three-, or even multi-photon states [10], as well as to independent photons [11]. These advancements underscore the versatility of SPDC-generated photons and their utility in quantum optics research.
Implications and Applications
Dispersion cancellation illustrates how entanglement can preserve the integrity of quantum states against material-induced distortions. This property is valuable for quantum communication, where precise timing is critical, and for quantum imaging, where maintaining narrow correlations enhances resolution. At Raicol Crystals, we specialize in crafting nonlinear crystals that enable such experiments, providing researchers with the tools to explore and harness these effects. For more information on our quantum solutions, visit our quantum components page, or contact us directly.
References
[1] Franson, J. D. “Nonlocal cancellation of dispersion.” Physical Review A 45, no. 5 (1992): 3126.
[2] Shapiro, Jeffrey H. “Dispersion cancellation with phase-sensitive Gaussian-state light.” Physical Review A—Atomic, Molecular, and Optical Physics 81, no. 2 (2010): 023824.
[3] Franson, J. D. “Lack of dispersion cancellation with classical phase-sensitive light.” Physical Review A—Atomic, Molecular, and Optical Physics 81, no. 2 (2010): 023825.
[4] Steinberg, A. M., P. G. Kwiat, and R. Y. Chiao. “Dispersion cancellation in a measurement of the single-photon propagation velocity in glass.” Physical review letters 68, no. 16 (1992): 2421.
[5] Steinberg, Aephraim M., Paul G. Kwiat, and Raymond Y. Chiao. “Dispersion cancellation and high-resolution time measurements in a fourth-order optical interferometer.” Physical Review A 45, no. 9 (1992): 6659.
[6] Baek, So-Young, Young-Wook Cho, and Yoon-Ho Kim. “Nonlocal dispersion cancellation using entangled photons.” Optics express 17, no. 21 (2009): 19241-19252.
[7] O’Donnell, Kevin A. “Observations of dispersion cancellation of entangled photon pairs.” Physical review letters 106, no. 6 (2011): 063601.
[8] Larchuk, Todd S., Malvin C. Teich, and Bahaa EA Saleh. “Nonlocal cancellation of dispersive broadening in Mach-Zehnder interferometers.” Physical Review A 52, no. 5 (1995): 4145.
[9] Agarwal, Girish S., and S. Dutta Gupta. “Filtering of two-photon quantum correlations by optical cavities: Cancellation of dispersive effects.” Physical Review A 49, no. 5 (1994): 3954.
[10] Nodurft, I. C., S. U. Shringarpure, B. T. Kirby, T. B. Pittman, and J. D. Franson. “Nonlocal dispersion cancellation for three or more photons.” Physical Review A 102, no. 1 (2020): 013713.
[11] Im, Dong-Gil, Yosep Kim, and Yoon-Ho Kim. “Dispersion cancellation in a quantum interferometer with independent single photons.” Optics Express 29, no. 2 (2021): 2348-2363.
Author: Ori levin
At Raicol, as a world leader in the manufacture of PPKTP, our goal is to support the growing quantum industry and drive it forward as much as we can.
To support this goal, Raicol is continuously cooperating with research centers and leading universities worldwide to provide them with advanced quasi-phase-matching crystals that help them accomplish innovative research. At times, this requires us to push the envelope to improve our fabrication techniques and bring new capabilities.
In the past few years, Raicol has been dealing with repeating requests for PPKTP with low absorption, higher pump powers, and requests for shorter poling periods. We found some of these requirements intriguing and challenging and started researching different solutions to tackle these issues.
One of the leading challenges our customers face is dealing with gray tracking.
Gray tracking is a phenomenon where optical beams, above the average power threshold, propagate through PPKTP crystal and create photorefractive damage that leaves opaque areas, making the crystal useless.
In unpolled KTP which is used for nonlinear conversions, Raicol solved this issue by developing the HGTR KTP which was designed to support much higher power densities, however, HGTR KTP cannot be easily polled, thus does not offer a solution for the quantum industry.
To tackle this problem, Raicol has started a research project to develop a new product that will support a higher power-frequency conversion process
(such as SPDC) but at the same time, will not compromise the PPKTP absorption parameters. During the research, we have emphasized optimizing the Raicol bulk KTP absorption.
Following two years of research, Raicol developed a version of HGRK KTP that can be polled and created the new version of ppKTP which we called SPPKTP. The SppKTP supports up to 6 times the power as a standard PPKTP and has about 40% less absorption (ppm) in the 532 nm wavelength .
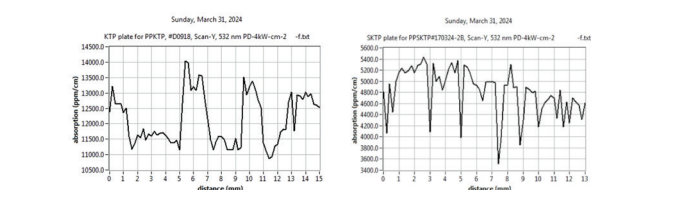
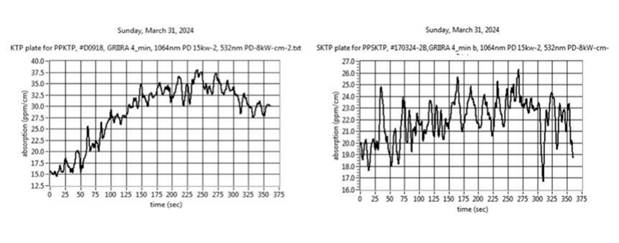
This technology finds numerous applications where even the smallest optical losses are highly detrimental or systems that can benefit from higher powers than typical KTP can support. Key examples include Optical Parametric Oscillators (OPOs), squeezed light sources, Quantum communications and quantum encryption (QKD), and high-power laser systems, where the high circulating power within the optical cavity demands an elevated optical damage threshold, exceptional crystal purity, and minimal losses to maintain efficient frequency conversion. In such systems, even minute losses can lead to reduced performance, thermal effects, and beam quality degradation.
In contrast, there are also applications operating at low power but requiring extremely low optical losses, such as systems with high Q-factor optical cavities. Here, losses directly influence the cavity linewidth, with narrow linewidths being essential for applications in high-precision metrology, laser stabilization, and quantum technologies.
Raicol’s SPPKTP crystals represent a significant advancement in nonlinear optical materials, driving innovation across diverse areas of photonics technology. Their breakthrough properties help unlock new possibilities in the field, from quantum applications to high-power laser systems
For detailed information about our sPPKTP crystals, download our product brochure or reach out to our team.
Have you already subscribed to our YouTube channel? Don’t miss out—subscribe now for exclusive content and updates from our company.
Author: Ronen Shekel
Quantum and classical ghost imaging
Ghost imaging, one of the first imaging techniques using quantum light, has captivated researchers since its inception in the mid-1990s. The concept of ghost imaging was first demonstrated in 1995 by Pittman et al. [1], using quantum light generated via spontaneous parametric down-conversion (SPDC), a nonlinear optical process that converts a pump photon into two lower-energy entangled photons.
In this experiment (Fig. 1a), the spatially entangled photons are separated, with one photon propagating through an object and being collected using a bucket detector with no spatial resolution, while the idler photon is sent to a multi-pixeled camera. By measuring coincidence events between the bucket detector and the camera, the object is reconstructed, despite the fact that the photons detected by the camera did not directly interact with it! This nonlocal characteristic led to the term “ghost imaging.”
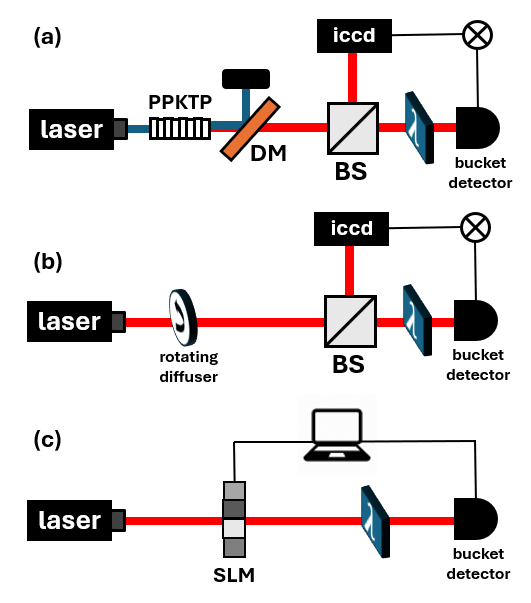
The crux of this technique lies in the spatial correlations of the two photons: areas that are blocked by the object will not reach the bucket detector, so coincidence events will not occur with the corresponding pixels in the camera. But one must remember: correlations do not necessarily imply entanglement! Does quantum entanglement play a critical role in this technique? This was the subject of a debate that lasted several years.
In [2], for instance, theoretical arguments were presented, suggesting that entanglement is intrinsic to ghost imaging. However, in 2002, Bennik et al. [3] demonstrated ghost imaging using two classically correlated beams, randomly deflected at different angles. Others explored similar classical correlations using a rotating diffuser [4], generating correlated pseudo-thermal light (Fig. 1b).
Computational ghost imaging
An important fruit of this debate was that of computational ghost imaging, proposed by Shapiro [5], and demonstrated by Bromberg et al. [6]. In this method, only a single detector is used, and the high-resolution camera is replaced by a computation of the propagating field which is shaped using a spatial light modulator (Fig. 1c). The key point here is that we know everything about the photon propagating to the camera, so we can utilize this knowledge computationally and get rid of the physical camera. The object image is obtained by correlating the intensities measured by the bucket detector with the calculated field at the object plane.
While this computational approach already simplified the experimental setup, researchers soon realized [7] that the number of measurements could be significantly reduced by leveraging modern image processing techniques, particularly those that exploit prior knowledge about the image structure. Remarkably, for most imaging tasks, such information exists: natural images are sparse, that is, they contain many coefficients close to or equal to zero when represented in an appropriate basis. This property of natural images is at the core of modern lossy image compression algorithms, such as JPEG. The field of compressed sensing exploits this sparsity/compressibility to reduce the number of measurements needed for faithful image recovery. Utilizing this technique reduces the number of measurements required for a faithful reconstruction by an order of magnitude.
Current state and future directions
The fact that computational ghost imaging uses only a single detector provides experimental evidence that pseudothermal ghost imaging does not inherently rely on nonlocal quantum correlations. It is now also recognized that the quantum and classical methods produce images of a similar resolution. The main advantage of utilizing quantum light for ghost imaging is found at low light levels, at which the quantum modality exhibits greater visibility and a greater signal-to-noise ratio [8]. This could be especially important when imaging samples that are sensitive to high light levels. Further details comparing the classical and quantum modalities may be found in [9].
Almost 30 years since its first demonstration, many extensions, applications, and modalities of ghost imaging are still being explored. Are you interested in ghost imaging with quantum light? Or perhaps want to explore other use cases for quantum entanglement? Please check out our PPKTP crystal and BBO crystals, used for generating entangled photons, and join the conversation!
[1] Pittman, Todd B., Y. H. Shih, D. V. Strekalov, and Alexander V. Sergienko. “Optical imaging by means of two-photon quantum entanglement.” Physical Review A 52, no. 5 (1995): R3429.
[2] Abouraddy, Ayman F., Bahaa EA Saleh, Alexander V. Sergienko, and Malvin C. Teich. “Role of entanglement in two-photon imaging.” Physical review letters 87, no. 12 (2001): 123602.
[3] Bennink, Ryan S., Sean J. Bentley, and Robert W. Boyd. ““Two-photon” coincidence imaging with a classical source.” Physical review letters 89, no. 11 (2002): 113601.
[4] Valencia, Alejandra, Giuliano Scarcelli, Milena D’Angelo, and Yanhua Shih. “Two-photon imaging with thermal light.” Physical review letters 94, no. 6 (2005): 063601.
[5] Shapiro, Jeffrey H. “Computational ghost imaging.” Physical Review A—Atomic, Molecular, and Optical Physics 78, no. 6 (2008): 061802.
[6] Bromberg, Yaron, Ori Katz, and Yaron Silberberg. “Ghost imaging with a single detector.” Physical Review A—Atomic, Molecular, and Optical Physics 79, no. 5 (2009): 053840.
[7] Katz, Ori, Yaron Bromberg, and Yaron Silberberg. “Compressive ghost imaging.” Applied Physics Letters 95, no. 13 (2009).
[8] Moreau, Paul-Antoine, Ermes Toninelli, Thomas Gregory, and Miles J. Padgett. “Imaging with quantum states of light.” Nature Reviews Physics 1, no. 6 (2019): 367-380.
[9] Erkmen, Baris I., and Jeffrey H. Shapiro. “Ghost imaging: from quantum to classical to computational.” Advances in Optics and Photonics 2, no. 4 (2010): 405-450.
Do you have a question? Our experts will be happy to hear from you and advise you on the best product for you. Contact Us.
Have you already subscribed to our YouTube channel? Don’t miss out—subscribe now for exclusive content and updates from our company.
Author: Yoad Michael
PPKTP Crystal is a quasi-phased-matched crystal that converts light of one wavelength into a different wavelength. The original purpose of this crystal was humble: It was designed to be an efficient frequency converter for laser system by second harmonic generation. However, with recent advances in quantum optics, the process of spontaneous parametric down conversion (SPDC) is now the dominating application of PPKTP.
SPDC is a process in which a strong pump beam is converted into correlated photon pairs, coined Signal and Idler. These correlations are the basis for various sources of quantum light, such as heralded single photons, time-energy or polarization entangled photon pairs, and squeezed light.
While it is convenient to consider only the quantum properties these light sources, the nonlinear properties of the interaction play a very significant role. For example, in PPKTP, the phase matching can be fully controlled such that: the signal and idler have the same polarization (type-0) or orthogonal polarization (type-2), same wavelength (degenerate) or separate wavelengths (nondegenerate), emitted along the direction of the pump beam (collinear) or at some angle (noncollinear). The type-0/type-2 is determined by the polling period and is something that needs to be determined at the manufacture stage, while degeneracy and collinearity can be fine-tuned by temperature (to avoid working at bizarre temperatures, it is advised to specify these parameters beforehand and adjust the polling period).
We can dive deeper into these differences. Polarization is not the only optical property that changes between type-0 and type-2 interactions; Spectral bandwidth, pair rate, and tolerance to temperature are also different. This is best illustrated by the wonderful work of the Ursin group [1], in which they compared type-0 and type-2 crystals as sources of polarization entangled photon pairs. As shown in Figure. 1, Type-0 is significantly broader than Type-2 and can be made nondegenerate by temperature tuning. In addition, the authors reported that the pair generation (per nm) of type-0 is about 10 times higher than that of type-2.
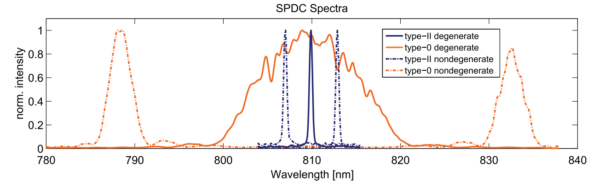
To make things a little more complicated, the dispersion and the length of the KTP Crystal also affects the spectral bandwidth and pair rate. Longer crystals generate more pairs at the cost of reduced spectral bandwidth, and signal/idler photons are much broader at telecom wavelengths (~1550) than they are at NIR (~810).
All these examples show that PPKTP is an extremely versatile component, and it is therefore important to first consider the needs of each application before choosing the right crystal. Below we present a few recent utilizations of PPKTP for various applications and provide our recommended crystal. We are proud to say that all this knowledge came from the brilliant researchers that use our PPKTP Crystals and shared their findings with the scientific community. Feel free to contact us if you think we are missing a key application or research work.
Boson Sampling and quantum interference
In Boson Sampling, quantum light is usually placed at the input of a large interferometer that includes multiple splitting and re-combining of beams. Boson Sampling relies on quantum interference (Hong-Ou-Mandel effect) and therefore benefits from high spectral purity. Special efforts were made by the Fedrizzi group for creating aperiodically polled crystals for high spectral purity at 1550nm [2], and a similar design was implemented by USTC’s photonic quantum supremacy experiment [3]. Recent quantum computing efforts by Xanadu [4] and QuiX [5] utilized PPKTP at the same wavelength region, due to both higher purity at these wavelengths and compatibility with peripheral platforms such as Silicon Nitride. Raicol has developed (through a collaboration with Prof. Ady Arie) a method for the design and manufacture of high spectral purity APKTP Crystals for Boson sampling and quantum interference close to the group velocity matching point of 1550nm.
Recommended Crystal: Type-2 APKTP or PPKTP at 775->1550. APKTP offers higher spectral purity while PPKTP offers higher pair rate.
Quantum Key Distribution
PPKTP plays a role in entanglement-based QKD as a source of polarization-entangled photon pairs. In this field there are many available options depending on whether the system is designed for free-space or fiber. In general, detector efficiency and the availability of 405nm lasers usually pushes these applications towards entanglement at 810nm [6, 7]. Type-2 crystals are easier to use because of their narrow linewidth, easy separation of the signal and idler with a polarizing beam splitter, and robustness to temperature, while type-0 crystals are broader and offer higher pair rate, making them good candidates for multiplexed QKD [8].
Recommended Crystal: Type-0 or Type-2 PPKTP at 405->810. Type-0 offers higher pair rate and spectral bandwidth, while Type-2 offers ease of usability.
Squeezed Light
Squeezed light usually utilizes the crystals in the strong pumping regime (unlike heralded single photons or polarization entanglement), and benefits from a strong nonlinear response, therefore making type-0 crystals the favorable option. Examples include the Furusawa group with 9dB of squeezing at 860nm [9], The Schnabel group has demonstrated 15dB and 13dB of squeezing at both 1064 and 1550nm [10, 11], and the Bowen group using the former for a demonstration of squeezing-enhanced microscopy [12]. Squeezed light can be generated anywhere from 780nm (390nm pump) to 3.8 microns and is a function of the exact specific application.
When choosing a crystal for squeezed light applications, the researcher should first decide if the squeezing is going to be generated in single-pass or in a cavity. For the former, a standard crystal suffices, while for an optimal parametric oscillator, monolithic or hemi-monolithic options are preferred [13].
Recommended Crystal: Type-0 ppKTP, optional hemi or fully monolithic.
Imaging With Undetected Photons
Imaging with undetected photons usually utilizes type-0 crystals with varying degeneracy. For fundamental research it is convenient to be able to detect both photons [14], while the Ramelow group generated a signal in the visible and idler at the mid-IR for microscopy applications [15].
Recommended Crystal: Type-0 PPKTP, with a period that is designed for nondegeneracy. No better example than Ramelow’s 660->800+3800.
We will try to update this list periodically, so visit this page every now and then!
Bibliography
[1] Steinlechner et al. “Efficient heralding of polarization-entangled photons from type-0 and type-II spontaneous parametric downconversion in periodically poled KTiOPO4”, JOSA B 31, 9, 2068-2076 (2014).
[2] Graffitti et al. “Independent high-purity photons created in domain-engineered crystals”, Optica 5, 5, 514-517 (2018).
[3] Zhong et al. “Quantum computational advantage using photons”, Science 370, 6523, 1460-1463 (2020).
[4] Madsen et al. “Quantum computational advantage with a programmable photonic processor”, Nature 606, 75–81 (2022).
[5] Taballione et al. “20-Mode Universal Quantum Photonic Processor”, arXiv:2203.01801.
[6] Yin et al. “Entanglement-based secure quantum cryptography over 1,120 kilometres”, Nature 582, 501–505 (2020).
[7] Mishra et al. “BBM92 quantum key distribution over a free space dusty channel of 200 meters”, Journal of Optics, 24, 7 (2022).
[8] Brambila et al. “Ultrabright Polarization-Entangled Photon Pair Source for Frequency-Multiplexed Quantum Communication in Free-Space”, arXiv:2205.10214.
[9] Takeno et al. “Observation of -9 dB quadrature squeezing with improvement of phase stability in homodyne measurement”, Optics Express 15, 7, 4321-4327 (2007).
[10] Vahlbruch et al. “Detection of 15 dB Squeezed States of Light and their Application for the Absolute Calibration of Photoelectric Quantum Efficiency”, Physical Review Letters 117, 110801 (2016).
[11] Schönbeck et al. “13 dB squeezed vacuum states at 1550 nm from 12 mW external pump power at 775 nm”, Optics Letters 43, 1, 110-113 (2018).
[12] Casacio et al. “Quantum-enhanced nonlinear microscopy”, Nature 594, 201–206 (2021).
[13] Ast et al. “High-bandwidth squeezed light at 1550 nm from a compact monolithic PPKTP cavity”, Optics Express 21, 11, 13572-13579 (2013).
[14] Gilaberte Basset et al. “Video-Rate Imaging with Undetected Photons”, Laser & Photonics Reviews 15, 6 (2021).
[15] Kviatkovsky et al. “Microscopy with undetected photons in the mid-infrared”, Science Advances 6, 42 (2020).
Do you have a question? Our experts will be happy to hear from you and advise you on the best product for you. Contact Us.
Have you already subscribed to our YouTube channel? Don’t miss out—subscribe now for exclusive content and updates from our company.
Author: Dr. Noa Voloch Bloch and Ori Levin
Difference Frequency Generation (DFG) and Sum Frequency Generation (SFG) are crucial processes in modern laser systems, allowing for the generation of new optical frequencies by mixing two distinct wavelengths. For professionals in industries such as defense, aerospace, medical, and industrial applications, selecting the right nonlinear crystal is critical to achieving optimal performance.
With over 50 years of experience in crystal growth, Raicol Crystals is at the forefront of providing high-quality nonlinear optical crystals and electro-optical components for laser systems worldwide. In this article, we will explore key aspects of DFG and SFG and how Raicol’s expertise in crystal manufacturing can significantly enhance the efficiency and precision of your laser systems.
1. What are Difference Frequency Generation (DFG) and Sum Frequency Generation (SFG) and How Do They Work? Difference Frequency Generation (DFG) is a nonlinear optical process in which two laser beams with different frequencies mix within a nonlinear crystal to produce a new output beam at the frequency difference between the two input beams. Conversely, Sum Frequency Generation (SFG) combines two input wavelengths to create a new beam at the sum of their frequencies. The crystals’ nonlinear susceptibility couples between the different laser input and create a new frequency output.
2. These techniques are essential for producing tunable sources of coherent radiation in the infrared, terahertz, and visible regions.
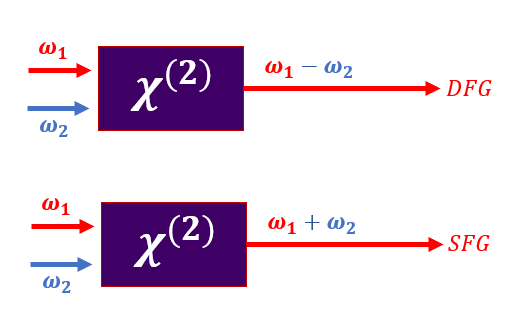
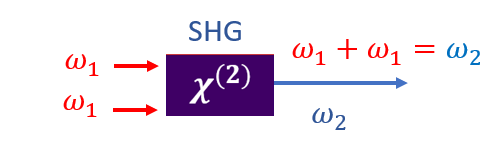
- Second Harmonic Generation (SHG) is a special case of Sum Frequency Generation (SFG) where two photons of the same frequency combine to produce a single photon with twice the frequency (or half the wavelength) of the input light. In this degenerate process, two identical photons from the fundamental beam are converted into one photon at the second harmonic through interaction with a nonlinear optical material.
The efficiency of this process depends critically on phase-matching conditions within the nonlinear crystal. SHG has become a widely used technique for generating new frequencies, particularly for accessing shorter wavelengths in laser systems and spectroscopic applications.
Raicol Crystals specializes in providing nonlinear crystals optimized for both DFG and SFG applications. By selecting the right material and engineering the crystal to meet specific wavelength requirements, Raicol ensures that DFG and SFG systems achieve high efficiency, precision, and stability. This makes their nonlinear crystals ideal for advanced laser systems requiring reliable and precise frequency control.
2. Which Nonlinear Crystals are Suitable for DFG and SFG?
- The efficiency of DFG and SFG processes depends significantly on the nonlinear crystal’s properties, including its nonlinear coefficient, damage threshold, and phase-matching capabilities. Commonly used nonlinear crystals for DFG and SFG include Potassium Titanyl Phosphate (KTP), Beta Barium Borate (BBO), and periodically poled lithium niobate (PPLN). These materials offer excellent nonlinear properties, high damage thresholds, and thermal stability in various wavelength ranges.
Raicol Crystals specializes in high-quality oxide-based nonlinear crystals, such as these, that are designed to handle high power, high efficiency, and thermal stability in laser systems. Additionally, Raicol’s ability to offer customized solutions means that customers can select the optimal material tailored to their specific DFG and SFG needs. Whether it’s for medical diagnostics, industrial applications, or defense systems, Raicol’s products ensure maximum performance in frequency generation applications.
3. How do Nonlinear Crystals Enhance DFG and SFG Efficiency?
- The efficiency of DFG and SFG heavily relies on the properties of the nonlinear crystal, such as its nonlinear coefficient, transparency range, and phase-matching capabilities. For maximum efficiency, the crystal must support the specific phase-matching conditions for the wavelengths of the input beams.
Raicol Crystals leverages over 50 years of expertise in crystal growth to provide customized solutions that enhance DFG and SFG efficiency. Their nonlinear crystals are optimized for high conversion efficiency, low losses, and the ability to handle high-power lasers. Raicol’s advanced manufacturing processes ensure that each crystal is precisely engineered to support the phase-matching conditions, allowing for superior performance in demanding applications.
Raicol’s tailored solutions enable clients to achieve the best possible output from their DFG and SFG systems, improving efficiency and ensuring reliable, high-quality results, particularly in critical industries like defense, aerospace, and medical diagnostics.
4. Why Choose Raicol Crystals for DFG and SFG Applications in Industrial and Defense Sectors?
- Raicol Crystals is a global leader in manufacturing nonlinear crystals, with deep expertise in the specific requirements of the defense and industrial sectors. Their advanced crystal solutions enable high-performance systems essential for applications like advanced radar, laser weapons, and high-resolution spectroscopy.
By offering customized nonlinear crystals that are engineered to meet the specific needs of these industries, Raicol ensures that its customers can achieve the precision and reliability required for mission-critical systems. Whether you’re designing next-generation defense technologies or enhancing industrial laser applications, Raicol’s nonlinear crystals provide the high performance necessary for success.
5. How Raicol Crystals Supports Medical Field with DFG and SFG Applications?
- In the medical field, DFG and SFG processes are used in non-invasive imaging techniques. The ability to generate precise wavelengths through these frequency generation methods enables improved resolution and sensitivity in medical imaging systems, making them valuable tools for diagnostics.
Raicol Crystals manufactures nonlinear optical crystals that are ideal for DFG and SFG applications in medical imaging. Their customized crystals ensure high frequency conversion efficiency, enhancing the performance of optical diagnostic devices. By optimizing the wavelength conversion process, Raicol’s crystals improve the clarity and accuracy of medical images, aiding in better diagnostic procedures and patient outcomes.
Conclusion: Why Raicol Crystals is the Optimal Choice for DFG and SFG Applications Difference Frequency Generation (DFG) and Sum Frequency Generation (SFG) play critical roles in many of today’s advanced optical technologies. For industries like defense, aerospace, medical, and industrial applications, the performance of DFG and SFG systems is intrinsically linked to the quality of the nonlinear crystals used.
Raicol Crystals, with over 50 years of expertise in crystal growth and a proven track record of providing high-quality nonlinear optical crystals, is the ideal partner for your DFG and SFG applications. Their commitment to precision, customized solutions, and high-performance products ensures that your frequency generation systems operate at peak efficiency and deliver optimal results.
Whether you’re enhancing the performance of existing laser systems or developing cutting-edge technologies, Raicol Crystals provides the knowledge, technology, and products needed to succeed.
Explore Raicol’s range of nonlinear optical crystals and electro-optical components to take your DFG \SFG systems to the next level.
Do you have a question? Our experts will be happy to hear from you and advise you on the best product for you. Contact Us.
Have you already subscribed to our YouTube channel? Don’t miss out—subscribe now for exclusive content and updates from our company.